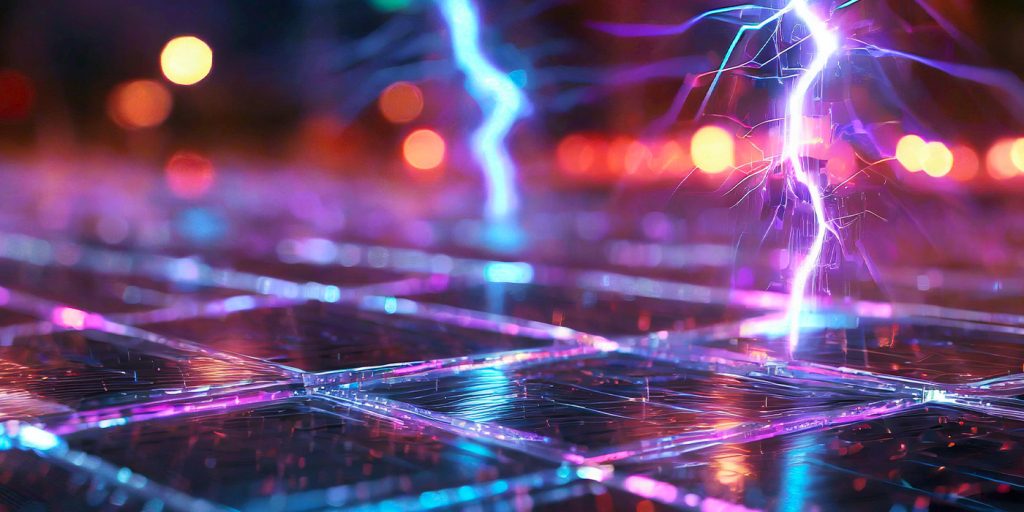
Glass, a highly functional material integral to modern technology, plays an active role in the world of electronics and energy. From the screens of our smartphones to the fibers that carry internet signals across oceans, glass is at the heart of many technologies that rely on its unique electrical properties.
This article aims to shed light on these properties—from dielectric constants to electrical resistivity—exploring how they are influenced by glass composition. We will delve into the science behind these properties and their diverse applications in today’s technology-driven world. This journey will reveal not just a material but a versatile and essential component of countless electrical and electronic innovations.
The Relationship Between Composition and Electrical Properties
Dating back to 1845, glass was initially chosen for its insulating capabilities in telegraph communications. Fast forward to today, over 360,000 different compositions of glass have been documented, each engineered for specific electrical, mechanical, and thermal properties to meet diverse technological needs. This broad spectrum of compositions is a testament to the material’s adaptability in electrical applications.
The following discussion will detail some of the commonly measured electrical properties of glass,2 examine the effects of compositional variations on these properties, and provide insight into the role of different components in enhancing or moderating these properties for advanced technological applications.
The Dielectric Constant
The dielectric constant is a critical factor in a material’s ability to store electrical charge under an electric field. As a dielectric material, glass acts as an electrical insulator, meaning that electric charges do not flow through it as they do in conductors like metals. Instead, when exposed to an electric field, the positive and negative charges within the glass are displaced—positive charges move towards the field, while negative charges move away. This charge separation, or polarization, reduces the internal electric field in the glass, enabling it to store charge.3
This dielectric behavior can be observed when glass is inserted between the plates of a parallel-plate capacitor. This augments the capacitance value, enhancing its ability to store opposing charges on each plate.3
The relationship between the composition of glass and its dielectric constant is an active research area. This relationship is complex, requiring an understanding of how the various elemental components within glass contribute to polarization.
Generally, glasses with higher dielectric constants tend to contain alkalis, alkaline earth, and heavy-metal cations like lead, bismuth, and tungsten,4 and research has revealed that altering the composition of glass can impact its dielectric constant. For instance, replacing silicon with aluminum in sodium aluminosilicate glasses, increasing tungsten oxide in sodium germanium borosilicate glasses, and adjusting copper oxide levels in borate zinc-fluoride glasses can raise the dielectric constant. On the other hand, adding rare earth oxides to alkali-free aluminoborosilicate glass can lower its dielectric constant.4,5,6,7
By manipulating the elemental makeup of glass, researchers and engineers can tailor its electrical characteristics to meet specific technological needs, paving the way for advanced and specialized uses in various industries.
Loss Tangent
When glass is exposed to an alternating electric field, a portion of the electrical energy is stored in line with its dielectric constant. However, not all energy is efficiently stored; some is inevitably lost, predominantly as heat, a phenomenon known as dielectric loss. The dielectric loss tangent of glass relates to this dissipation of electrical energy and can result from various physical processes, including electrical conduction, dielectric relaxation, and dielectric resonance.8,9
For applications like high-frequency electronic circuits, where minimizing energy dissipation is crucial, the composition of the glass must be meticulously tailored to achieve a low loss tangent. This careful adjustment ensures that the glass performs optimally, avoiding unnecessary energy waste.
While it has been observed that the levels of impurities in glass can impact dielectric loss, in the case of borosilicate (BRS) glass-reinforced PTFE composites used for microwave substrate applications, researchers found that decreasing BRS filler size can reduce the loss tangent.10,11 This finding underscores the importance of material composition and structure in achieving desired electrical properties for specific technological applications.
Conductivity and Resistivity
Conductivity and resistivity are also fundamental properties, especially with regard to electronic applications. Put simply, electronic conductivity involves the transmission of electrical charge by electrons, which are negatively or positively charged electron holes. Glass, characterized by its covalent and ionic bonding structure, typically shows conductivity levels far lower than those in metals, making it an ideal insulator.
Notably, two glass families exhibit significant electronic conduction:
- Oxide glasses containing high amounts of transition metal oxides exhibit enhanced conductivity. These oxides introduce unpaired electrons, facilitating electron flow.
- Chalcogenide and tetrahedral glasses, where the inherent covalent bonding contributes to their conductive properties.12
By adjusting specific elements within the glass, such as incorporating elements with free electrons or modifying the bonding structures, manufacturers can tailor glass to have specific conductivity and resistivity characteristics. This ability to customize glass properties makes it versatile for various electronic applications, from insulators to conductive components.
Applications
Glasses with low dielectric constants are crucial in applications where minimizing electrical losses, signal distortion, or interference is essential. For this reason, they are used in high-performance microelectronic systems, such as substrates in semiconductor packaging and in thick-film resistors. On the other hand, glasses with high dielectric constants are important for high-magnitude capacitors and multilayer dielectrics.4,14
The exploitation of electronic conductivity in glass has also opened up a realm of opportunities in modern technology. Notably, the photoconductivity of selenium and arsenic-selenium glasses, driven by electronic conduction, has been pivotal in revolutionizing the photocopying process.
Moreover, certain glasses exhibiting electronic conductivity can dynamically switch between insulator and semiconductor states. This unique property has been applied in the development of computer memory devices, showcasing the versatility of glass in electronic applications. The photovoltaic characteristics of amorphous hydrogenated silicon, a product of its electronic conduction, also form the cornerstone of solar cell technology.12
Overall, the applications of glass with electrical properties are diverse and continually expanding, driven by ongoing research. This progress highlights the pivotal role that the electronic conductivity of glass plays across multiple high-tech industries, showcasing its versatility and importance in shaping modern technology.
How can Mo-Sci help?
Meticulous calibration and consideration of glass composition are crucial for accurate and meaningful measurements of the aforementioned electrical properties. Mo-Sci specializes in creating high-performance sealing glasses suited for a wide range of applications. Our expertise extends to customizing solutions, including the production of specialized glass substrates and ultra-pure glass frit.
For inquiries on sealing solutions or custom development services, contact the Mo-Sci team today.
References and Further Reading
- Chen, J., et al. (2017) Generation of shock lamellae and melting in rocks by lightning-induced shock waves and electrical heating. Geophysical Research Letters. doi.org/10.1002/2017GL073843
- Jones, R. (2010) An Incomplete History and Timeline of the Electric Telegraph and the CD 731 Compromise Insulator. Available at: https://www.insulators.info/shows/handouts/cd_731.pdf
- Al-Amoudi, M.A. (2020) Determining Dielectric Constants of Glass and Thin Film Using a Parallel Plate Capacitor. International Journal of Applied Science and Engineering Review. ISSN: 2582-6271.
- Hsieh, C., et al. (1996) Correlation between dielectric constant and chemical structure of sodium silicate glasses. Journal of Applied Physics. doi.org/10.1063/1.363824
- Mundher, M. (2023) Tungsten oxide effects on conductivity, dielectric parameters, and density of sodium germanium borosilicate glass. Journal of Materials Science: Materials in Electronics. doi.org/10.1007/s10854-023-10280-6
- Shaaban, S.M., et al. (2023) Influence of Copper Ions on the Structural, Mechanical, Radiation Shielding and Dielectric Properties of Borate Zinc-Fluoride Glasses. Journal of Electronic Materials. doi.org/10.1007/s11664-023-10564-x
- Zhang, L., et al. (2020) Influence of rare earth oxides on structure, dielectric properties and viscosity of alkali-free aluminoborosilicate glasses. Journal of Non-Crystalline Solids. doi.org/10.1016/j.jnoncrysol.2020.119886
- Poplavko, Y.M. (2019) Chapter 7 – Dielectrics. Electronic Materials: Principles and Applied Science. doi.org/10.1016/B978-0-12-815780-0.00007-4
- Sebastian, M.T. (2008) Chapter Two – Measurement of Microwave Dielectric Properties and Factors Affecting Them. Dielectric Materials for Wireless Communication. doi.org/10.1016/B978-0-08-045330-9.00002-9
- Rodriguez-Cano, R., et al. (2023) Broadband Characterization of Silicate Materials for Potential 5G/6G Applications. IEEE Transactions on Instrumentation and Measurement. doi.org/10.1109/TIM.2023.3256463
- Alhaji, I.A., et al. (2021) Effects of Particle Size on the Dielectric, Mechanical, and Thermal Properties of Recycled Borosilicate Glass-Filled PTFE Microwave Substrates. Polymers. doi.org/10.3390/polym13152449
- Varshneya, A.K., et al. (2019) Chapter 16 – Electronic Conduction. Fundamentals of Inorganic Glasses (Third Edition). doi.org/10.1016/B978-0-12-816225-5.00016-X
- Mo Sci. (2022) Applications of Thin and Thick Glass Films. Available at: https://mo-sci.com/applications-of-thin-and-thick-glass-films/ (Accessed on 17 November 2023).
- Li, J.C., et al. (2012) Characterization of Semiconductor Surface Conductivity by Using Microscopic Four-Point Probe Technique. Physics Procedia. doi.org/10.1016/j.phpro.2012.03.568